[Image above] The three main classes of oxide cathodes, discovered by John Goodenough’s group in the 1980s. Credit: Manthiram, Nature Communications (CC BY 4.0)
Since Sony first commercialized the technology in 1991, lithium-ion batteries have revolutionized our society.
Li-ion batteries’ higher energy density compared to other rechargeable battery systems made them key in advancing portable electronic devices and, more recently, electric vehicles and renewable energy storage. Their impact on society is so notable that three scientists central to the development of Li-ion batteries won the Nobel Prize in Chemistry last year.
One of those scientists is John Goodenough, professor and Virginia H. Cockrell Centennial Chair in Engineering at the University of Texas at Austin. We discussed his history and the Nobel Prize win in a CTT last October.
Though we discussed briefly his and the other scientists’ contributions to Li-ion battery development, we did not dive deeply into the science. But a new review article by ACerS Fellow Arumugam Manthiram does just that.
Manthiram, professor and Cockrell Family Regents Chair in Engineering at UT Austin, has known Goodenough since 1985, when he joined Goodenough’s lab at the University of Oxford (United Kingdom) for postdoctoral research. When Goodenough accepted a position at UT Austin in the United States, he persuaded Manthiram to come with him so they could continue their research on oxide cathodes.
Since moving to UT Austin, Manthiram has continued work on oxide cathodes but has worked on many other batteries and fuel cells as well. “In addition to lithium-ion batteries, my group has been focusing on a wide range of battery chemistries, including lithium-sulfur, sodium-sulfur, sodium-ion, multivalent-ion, all-solid-state, and redox flow batteries,” he says in an email. “With fuel cells, my group has done work on electrocatalysts and membranes for proton exchange membrane fuel cells and direct methanol fuel cells.”
Manthiram had the idea to write a review article on the development of oxide cathodes for Li-ion batteries for a long time. “I felt that such an article will be informative for the community to know how all the practically viable cathode development occurred in the 1980s with a profound basic science investigation,” he says.
Last summer, Manthiram finally had his chance. “I discussed [the review article] in person with the Nature Communications editor and he invited me to submit the article to Nature Communications,” he says. “As I was busy, it took a while and then I prepared it during December 2019.”
The final nine-page paper is summarized below.
Discovery of oxide cathodes
M. Stanley Whittingham of Binghamton University, one of the three Nobel Prize winners, laid the groundwork for rechargeable Li-ion batteries in the 1970s by discovering titanium disulfide that has a molecular structure which can house (intercalate) lithium ions. However, TiS2-based batteries faced two major issues.
“First, the cell voltage was limited to <2.5 V, limiting the energy density. Second, dendrite growth on lithium-metal anodes during cell cycling caused internal shorting and presented a fire hazard,” Manthiram explains in the paper.
In the 1980s, Goodenough’s group at the University of Oxford explored cathodes made of oxides to see if such materials would increase cell voltage.
Cell voltage is determined by the energy difference between redox energies of the anode and cathode. So to achieve higher voltage, the cathode energy should be as low as possible, while the anode energy should be as high as possible.
Based on fundamental knowledge of transition-metal oxides, Goodenough knew the top of the sulfur energy band lies at a higher energy than the top of the oxygen energy band. So using oxides for the cathode would lower the cathode energy, thus increasing cell voltage.
This basic idea led Goodenough’s group to discover three classes of oxide cathodes—layered oxides, spinel oxides, and polyanion oxides.
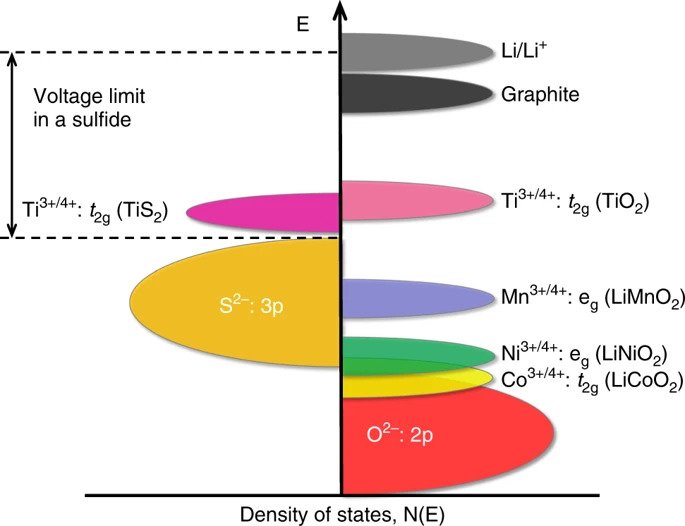
Positions of redox energies relative to the top of the anode (p bands). The top of the sulfur 3p band limits cell voltage to less than 2.5 V. In contrast, the lower-lying oxygen 2p band increases cell voltage substantially to about 4 V. Credit: Manthiram, Nature Communications (CC BY 4.0)
Three classes of oxide cathodes
The discovery of three oxide cathode classes involved three visiting scientists from three different parts of the world— Koichi Mizushima from Japan, Michael Thackeray from South Africa, and Manthiram (from India).
Layered oxides
Mizushima worked on layered LiCoO2, the first oxide cathode investigated. This cathode solved two major challenges associated with the sulfide cathodes pursued in the 1970s.
“It enabled not only a substantial increase in the operating voltage from <2.5 V to ~4 V but also the assembly of a cell without the need to employ a metallic lithium anode,” Manthiram writes.
Unfortunately, the cobalt energy band overlaps with the oxygen energy band, which leads to a release of oxygen from the crystal lattice on charging more than 50%. “Therefore, despite good electrochemical performance, the practical capacity of LiCoO2 is limited to ~140 mA h g–1,” Manthiram writes.
Since then, several layered LiMO2 oxides (M = 3d transition metals) have been investigated, including LiMnO2 and LiFeO2. However, they face numerous disadvantages, including structural changes during charge–discharge and charging difficulties. Instead, research in the past couple of decades has focused on substitution of cobalt with manganese and nickel to create layered cathodes with three transition-metal ions (NMC, nickel-manganese-cobalt).
Spinel oxides
Thackeray worked on the spinel LiMn2O4, the second class of cathode discovered. Compared to layered LiCoO2, LiMn2O4’s good 3D structural stability along with high electrical and lithium-ion conductivity offered even faster charge–discharge characteristics and a high operating voltage of 4 V with a practical capacity of just under 130 mA h g–1.
However, one critical issue with LiMn2O4 is dissolution of manganese in the presence of H+ ions (acidity) in the electrolyte. “Mn dissolution and its migration to the anode severely poison the graphite anode and limit the cycle life of lithium-ion cells,” Manthiram writes. Fortunately, substituting a small amount of lithium for manganese “perturbs the long-range Mn–Mn interaction/contact, frustrates the Mn3+ disproportionation reaction, reduces Mn dissolution, and thereby improves the cyclability,” he adds.
Spinel oxides are limited to the compositions LiM2O4 (M = titanium, vanadium, or manganese) because of difficulty in stabilizing the high oxidation states by conventional high-temperature synthesis. Of these, only the manganese-based spinel makes a suitable cathode. There have been efforts to prepare both LiCo2O4 and LiNi2O4 spinel oxides, but such attempts result in either incomplete transformation to spinel-like phases or loss of oxygen.
Polyanion oxides
Manthiram worked on polyanion oxides, the final class of oxide cathodes. He had previously worked with polyanion oxides during his Ph.D. in India and used that work to prepare the polyanion oxides Fe2(MoO4)3 and Fe2(WO4)3.
When compared to simple oxides, Manthiram found these polyanion oxides exhibited increased operating voltage. However, the rise of high-temperature copper oxide superconductors in the 1980s led Manthiram and Goodenough away from studying polyanion oxides for a while. It wasn’t until the commercialization of lithium-ion batteries with LiCoO2 cathode in 1991 that they refocused on polyanion oxides, specifically ones with phosphate groups (a topic they initially had Ph.D. student Geeta Ahuja explore).
Today, polyanion oxides, especially ones with phosphate groups, have become appealing cathodes for Li-ion or sodium-ion batteries because of the ability to increase voltage drastically (up to about 5 V). Compared to layered and spinal oxide cathodes, “the polyanion oxide class with sulfates, phosphates, and silicates has become diverse … in terms of versatility and number of materials,” Manthiram writes.
The future of oxide cathodes
Among the three classes of oxide cathodes, layered oxides are the favorite candidates—at least in the near term—for their high gravimetric and volumetric energy densities. However, as industry continues with large-scale deployment of Li-ion batteries for electric vehicles and energy storage, “cost and sustainability are becoming critical,” Manthiram writes.
In the conclusion, Manthiram previews some of the research taking place currently to advance Li-based battery technology, particularly lithium-sulfur batteries. Ultimately, he concludes that “innovative synthesis and processing approaches along with advanced characterization methodologies and computational analysis could aid the discovery of new materials as we continue our journey to realize a cleaner, more sustainable planet.”
The open-access paper, published in Nature Communications, is “A reflection on lithium-ion battery cathode chemistry” (DOI: 10.1038/s41467-020-15355-0).
Author
Lisa McDonald
CTT Categories
- Education
- Electronics
- Energy