[Image above] (A) High yield electrolytic synthesis of carbon nanostructures from dissolved air or smokestack concentrations of carbon dioxide in molten lithium carbonates. During CO2 electrolysis, transition metal deposition controls the nucleation and morphology of the carbon nanostructure. (B,C) Diffusion controls the formation of either nanotube (C: as grown from natural abundance CO2) or nanofiber (B: from 13C) morphologies. (D–F) Electrolytic oxide controls the formation of tangled (D: high Li2O) or straight (F: no added Li2O) nanotubes. Credit: Ren and Licht, Scientific Reports (CC BY 4.0)
During its presidency of the Group of Twenty (G20) in 2020, Saudi Arabia launched the concept of the circular carbon economy as a framework for reducing emissions to a level consistent with the goals of the Paris Agreement. This framework, which was endorsed by both G20 leaders and energy ministers, advocates for the use of techniques and technologies from the four Rs—reduce, recycle, reuse, and remove—to manage carbon emissions.
While the concept purports to embrace all four Rs, implementation of the circular carbon economy to date has very obviously focused on some Rs while neglecting others.
For example, many governments have funded projects on carbon capture and storage technologies, and some of these technologies have begun full-scale demonstrations. These technologies fulfill the “remove” pillar of the circular carbon economy, but far fewer projects have pursued commercializing the “recycle” or “reuse” of this stored carbon.
Projects that do recycle or reuse the stored carbon often convert it into hydrocarbon fuels, such as methane and ethanol, or other chemicals. However, “Many of the products made this way only briefly delay carbon’s journey into the atmosphere—fuels are burnt, products made from chemicals degrade, and the CO2 consumed during their creation is released again,” a Nature news feature explains.
Recycling the carbon emissions into longer lasting products, such as polymers and concrete, is a step toward maximizing climate benefits. But it would be even more environmentally beneficial if we could break carbon dioxide down completely into solid carbon and oxygen gas. Then we could extract pure carbon products, such as graphite and carbon nanomaterials, and avoid the risk of re-releasing carbon dioxide into the atmosphere.
However, carbon dioxide typically separates into carbon monoxide and oxygen when split. Extracting pure carbon is traditionally an energy-intensive process that relies on burning fossil fuels.
Recent studies have shown that molten salt electrochemical conversion may offer an economic and relatively clean way to extract pure carbon products from carbon dioxide. In this process, carbon dioxide gas is readily absorbed by dissolved O2− ions in the molten salt, and the subsequently formed CO32− ions can then be reduced into solid carbon and oxygen gas following application of sufficient voltage.
In a recently published open-access paper, researchers from the University of Science and Technology Beijing summarize the successes and challenges of the molten salt electrochemical conversion process. Below are some highlights from their 17-page review.
Molten salts for electrochemical reduction of carbon dioxide
Molten carbonates and molten chlorides are typically used in the molten salt electrochemical conversion process because of their high solubility of O2− ions.
For molten carbonates, most studies use molten alkali metal carbonates, lithium carbonate, sodium carbonate, and potassium carbonate due to their superior stabilities under high working temperatures.
For molten chlorides, most studies use calcium-chloride-based and lithium-chloride-based salts due to the relatively high solubility of O2− ions in these systems.
Managing the competing carbon monoxide evolution reaction
For the reduction of CO32− ions into solid carbon, there are generally two accepted pathways: single-step reduction and two-step reduction.
The single-step reduction involves a direct four-electron transfer, splitting CO32− ions directly into solid carbon and O2− ions.
The two-step reduction involves the reduction of CO32− ions to CO22− ions and subsequent reduction of CO22− ions to solid carbon and O2− ions. However, the CO22− ions are unstable and may decompose into carbon monoxide gas and O2− ions.
The evolution reaction of carbon monoxide competes with the reaction for carbon deposition in molten salts. As seen in the graph below, the carbon monoxide evolution reaction is thermodynamically easier than the carbon deposition reaction when working temperature is higher than 970°C in molten Li2CO3. Thus, conducting the molten salt electrochemical conversion process at lower temperatures is beneficial to minimizing carbon monoxide evolution.
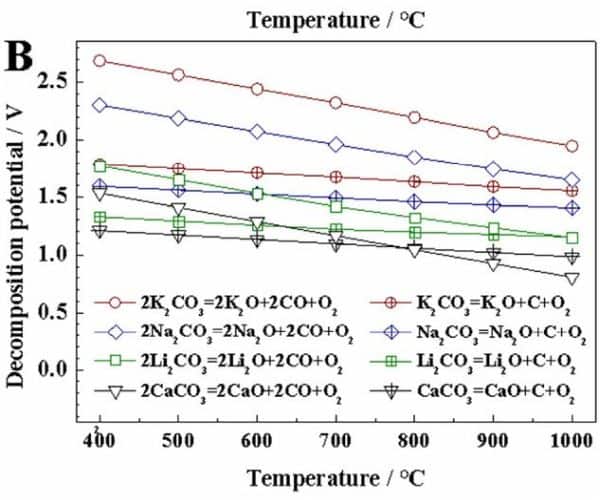
Thermodynamic calculation of the decomposition voltages for carbon monoxide evolution and carbon deposition. The calculations are conducted assuming all the components are in standard state. Data from HSC software. Credit: Zhu et al., Exploration (CC BY 4.0)
The reduction of carbon dioxide in molten salt can also be tuned by regulating the activity of O2− ions. For example, Hu et al. (here and here) reported the “buffer effect” provided by borate (BO2−) ions in molten LiCl─Li2CO3. The added BO2− ions can absorb O2− ions to form BO33− ions, which significantly decrease the activity of O2−. The formed BO33– ions can then react with CO2 to regenerate BO2− and release CO32−. The decreased activity of O2− positively shifts the potentials of carbon deposition and carbon monoxide evolution, which allows the reactions to occur at low temperatures.
Transforming amorphous carbon products into graphitic carbon materials
The molten salt electrochemical conversion process results in carbon products containing large amounts of amorphous carbon. To convert the amorphous carbons into graphitic carbons (e.g., nanotubes, nanofibers), small amounts of additives or catalysts typically are used, but these additions may contaminate the final products.
Recently, a strategy was proposed for transforming amorphous carbons into graphitic carbons without using any catalysts. This electrochemical graphitization process involves two steps: the electrochemical removal of oxygen through cathodic polarization, and the rearrangement of carbon atoms into a graphite crystal lattice.
The electrochemical graphitization process is applicable to various amorphous carbon feedstocks, from “soft carbons” to “hard carbons.” The typical “hard carbon,” carbon black, which cannot be graphitized by the conventional high-temperature treatment, was successfully converted into nanoflake graphite by cathodic polarization.
Various studies have shown that the graphitic carbon materials prepared in molten salts exhibit excellent electrochemical performance when tested as electrode materials for energy storage (e.g., batteries and supercapacitors).
Future directions for research
If molten salt electrochemical conversion is to be scaled-up for commercial production of graphitic carbons, the energy consumption of the process and the quality of the carbon products need to be compared to other synthesis routes.
Additionally, more needs to be learned about the reduction mechanisms that turn carbon dioxide into solid carbon and oxygen gas. Very limited information can be obtained in situ by present electrochemical techniques (e.g., cyclic voltammetry and square wave voltammetry tests). So, in-situ techniques designed for high-temperature molten salts, such as high-temperature Raman and computed tomography, need to be further developed.
The open-access paper, published in Exploration, is “Molten salt electro-preparation of graphitic carbons” (DOI: 10.1002/EXP.20210186).
Author
Lisa McDonald
CTT Categories
- Education
- Environment