[Image above] A fast CCD camera (visible wavelength) image of plasma in the EAST Tokamak shows plasma impurity behaviors after t = 4.5 s. These instabilities can cause tungsten-armored walls to erode—how can scientists avoid that? Credit: EAST Tokamak, Wikimedia (CC BY 3.0)
When I was an intern for the ATLAS Experiment at CERN in the summer of 2018, I had the opportunity to visit the École Polytechnique Fédérale de Lausanne (EPFL), one of the two Swiss Federal Institutes of Technology in Switzerland. My goal in visiting EPFL was simple—to see the TCV Tokamak.
A tokamak is a magnetic confinement device that is a leading candidate for a practical fusion reactor. Tokamaks are torus (doughnut) shaped and use powerful magnetic fields to contain hot plasma. Fusion occurs when electric currents are pulsed over short time intervals in the plasma.
Research on tokamaks began in the 1950s, and by the 1970s dozens of tokamaks were in use experimentally around the world. However, these machines were unable to achieve all conditions necessary for practical fusion. In the 1990s, a new series of tokamaks were designed that used a mixture of deuterium and tritium (two isotopes of hydrogen) as fuel. This design is the one most commonly researched today.
The TCV Tokamak is one of five tokamaks currently funded under Horizon 2020, the European Union’s €77 billion research and innovation funding program coming to an end this year. The research conducted on these five tokamaks helps scientists prepare for the giant International Thermonuclear Experimental Reactor (ITER) Tokamak currently under construction in the south of France.
One question researchers are investigating is how to control fusion reactions for longer time periods. When current is pulsed through a tokamak’s plasma to cause fusion, the current makes the plasma susceptible to various instabilities that can disturb plasma confinement. Thus, currents are generally pulsed for less than a minute (in the case of TCV, only 1–2 seconds). The ITER Tokamak, however, is expected to have pulse durations of 400–600 seconds (about 6–10 minutes), which means understanding what causes plasma instabilities and controlling for them is of utmost importance.
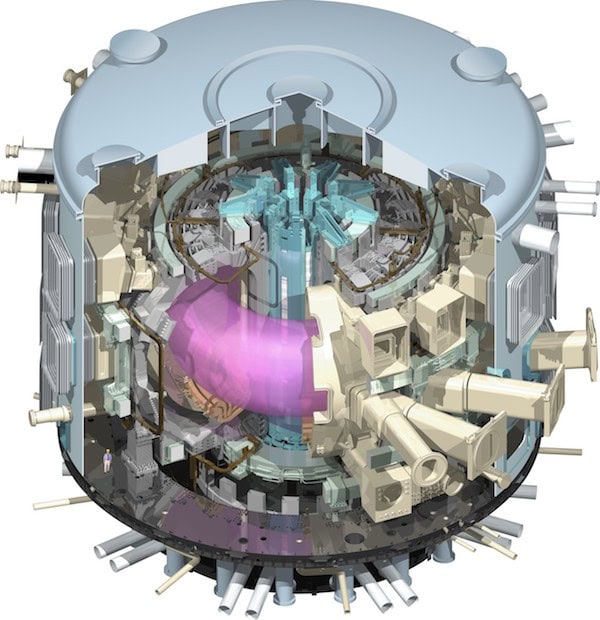
The ITER Tokamak will be nearly 30 meters tall, weigh 23,000 tonnes, and consist of an estimated one million parts. Credit: US ITER
A common plasma instability in tokamaks is large, periodic bursts of plasma known as edge localized modes (ELMs). ELMs lead to a significant expulsion of heat and particles against the divertor, a device within a tokamak that minimizes plasma contamination, extracts waste materials, and protects surrounding walls from thermal and neutronic loads.
Divertors traditionally were lined with heat-resistant carbon materials for protection. However, carbon is prone to react with tritium, a radioactive isotope of hydrogen in tokamak fuel, causing the carbon to trap tokamak fuel like a sponge. For ITER and future commercial fusion reactors, the metal tungsten instead will armor divertors, as it is less reactive with tritium.
While tungsten is superior to carbon during normal tokamak operation, tungsten has its own Achilles heel—ELMs.
When ELMs cause highly energetic particles to strike tungsten-armored divertors, the tungsten erodes. If ITER operates as planned for extended periods, ELMs are likely to occur, causing erosion of the tungsten-armored divertor.
“This motivates developing a validated understanding of the physical mechanisms which drive the gross and net erosion of tungsten divertor components during ELMs,” researchers write in a recent paper.
The researchers come from various universities around the United States and Canada and from the energy company General Atomics in San Diego. In this paper and an earlier one published in 2018, they investigate how ELMs cause tungsten to erode through models and experiments conducted on the DIII-D, a tokamak operated by General Atomics for the U.S. Department of Energy.
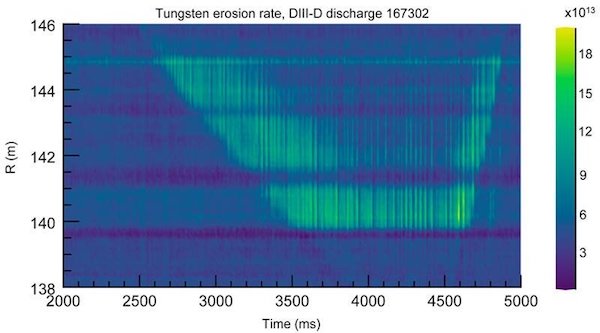
Measurements of the intensity of line radiation emitted by tungsten atoms eroding from the region of highest heat and plasma flux on the divertor. Credit: Tyler Abrams, General Atomics
In the 2018 paper, the researchers developed a Fundamenksi-Moulton “free-streaming” model (FSM) for the dynamics of divertor density, particle flux, and heat flux during ELMs. “This model depends only on inter-ELM pedestal and divertor conditions and, crucially, incorporates particle recycling,” they write.
Particle recycling refers to the circular process by which plasma particles leave the plasma, hit the wall, and are reemitted from the wall and subsequently re-ionized within the plasma. “Without recycling the plasma content would decay within the global particle confinement time τp after the external gas flux has been turned off,” explains a Max Planck Institute for Plasma Physics article.
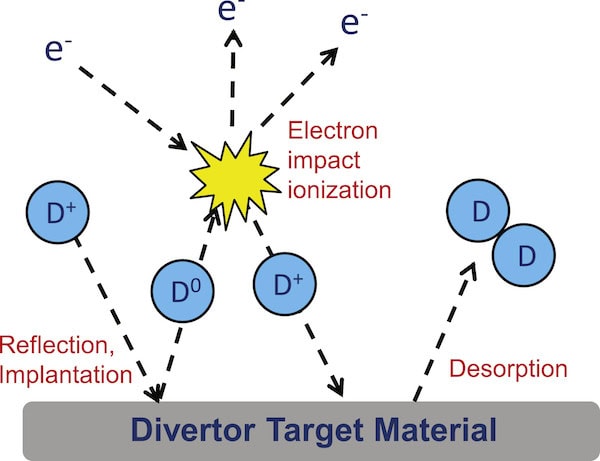
Diagram of the divertor particle recycling processes. Credit: Abrams et al., Nuclear Materials and Energy (CC BY-NC-ND 4.0)
The free-streaming plus recycling model (FSRM), experimentally validated using the DIII-D tokamak, “reinforces the conclusion that large, unmitigated ELMs have the potential to produce substantial tungsten erosion in ITER due to the high physical sputtering yields of energetic free-streaming pedestal ions and impurities,” the researchers write in the 2018 paper.
The more recent paper, published in June 2019, further investigates the FSRM developed in 2018 by testing it against an extensive array of DIII-D experimental scenarios.
“The FSRM framework reveals that W [tungsten] sputtering during ELMs is dependent almost entirely on the density and temperature of the main ions and impurities at the top of the pedestal,” the researchers write. “The dependence on divertor conditions is relatively weak, entering only through the divertor temperature which determines the sheath potential and, thus, regulates the physical sputtering yield of recycling ions.”
A DOE press release summarizes what these findings indicate about tungsten erosion in tokamaks. “[It] was found that nearly all the damage to the armor will be done by the deuterium and tritium fuel ions because they will be very energetic in ITER,” it says, “while the expected levels of beryllium impurity will not cause much erosion of the tungsten divertor.”
Based on these results, the researchers suggest that tungsten erosion may be reduced by maximizing the concentration of recycling particles near the tokamak wall while simultaneously minimizing the intensity of ELM bursts. How to accomplish these things, though, will require more studies.
Ceramics in tokamaks
Although metal trumps ceramics when it comes to the divertor armor, ceramics still play a crucial role in other parts of the tokamak, including
- The electrical transformer—the electrical transformer relies on long ceramic insulators called “bushings” to deliver current to the transformer.
- The fuel—tritium for the fuel is produced when neutrons escaping the plasma interact with lithium contained in the blanket (modules covering the inner walls of the vacuum vessel). ITER scientists are considering using ceramic pebbles to contain lithium in the blanket.
- Magnetic diagnostic sensors—the European Domestic Agency successfully tested prototypes of a specific type of magnetic diagnostic sensor based on low-temperature cofired ceramics, which will be installed in the ITER vacuum vessel (the heart of ITER).
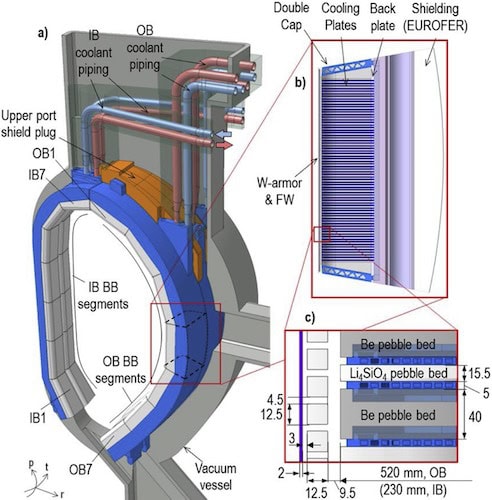
DEMO helium-cooled pebble bed design. Part c (bottom right) details the ceramic breeder and beryllium multiplier elements in the breeding blanket region. Credit: Federici et al., Fusion Engineering and Design (CC BY-NC-ND 4.0)
The 2018 paper, published in Nuclear Materials and Energy, is “Experimental validation of a model for particle recycling and tungsten erosion during ELMs in the DIII-D divertor” (DOI: 10.1016/j.nme.2018.10.011).
The 2019 paper, published in Physics of Plasmas, is “Impact of ELM control techniques on tungsten sputtering in the DIII-D divertor and extrapolations to ITER” (DOI: 10.1063/1.5089895)
Author
Lisa McDonald
CTT Categories
- Energy
- Modeling & Simulation