[Image above] Traditionally, only the edges of graphene sheets were considered to have electrocatalytic properties. But defects can impart such properties to the entire surface as well—the trick is identifying which defects do so best. Credit: Daria Sokol, MIPT Press Office
Let’s talk about defects.
Defects often are seen as something bad—a flaw, a weakness, a shortcoming. But in materials science, defects are normal—and commonly good.
When a material structure has defects, which most do, it means the structure is irregular. And these irregularities often determine the material properties, such as conductivity, mechanical strength, ductility, and more.
When scientists understand how defects and properties relate, they can alter defects to gain control over a material’s properties, thus making defects a very useful tool in material design.
Tons of research focuses on better understanding material defects, such as a study we covered last year on the difference between strontium and barium titanate defects. One material in which defects remain relatively unexplored, however, is graphene.
The large unknowns concerning graphene defects stem largely from how researchers traditionally understand graphene structure. A single layer of graphene is considered to have two different structural regions:
- The basal plane, or the surface parallel to the graphene plane consisting of conjugated sp2 carbon atoms,
- The edge plane, or the surface perpendicular to the graphene plane consisting of a one-atom thick line of carbon atoms with dangling bonds.
When layers of graphene are stacked to form graphite, the step edge refers to the perpendicular surface consisting of all edge planes.
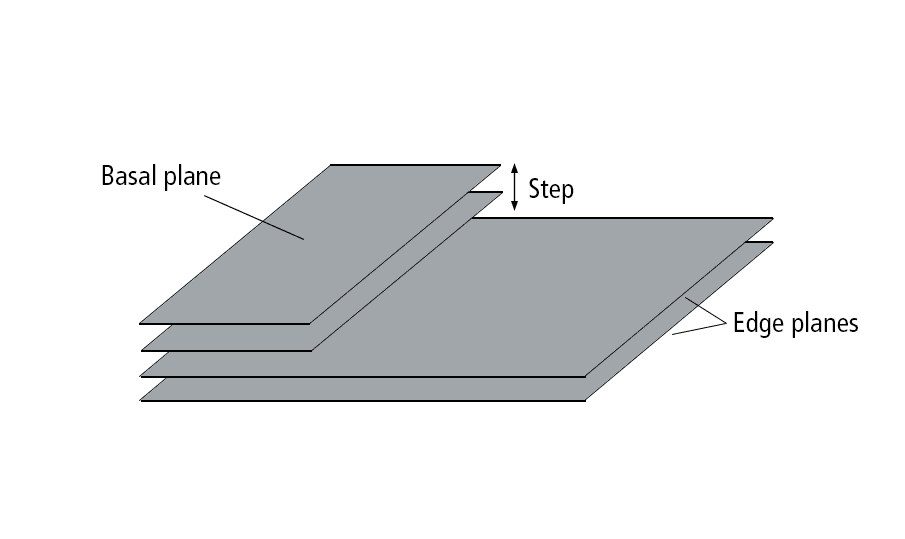
Illustration of graphite structure. Credit: ACerS
Traditionally, the step edge was considered much more reactive than the basal plane in graphite because of dangling bonds along the step edge, leading researchers to assume the basal surface in graphite-based electrodes had relatively low or even no electroactivity. However, recent investigations of pyrolytic graphite have shown otherwise.
Pyrolytic graphite refers to graphite with some covalent bonding between its graphene sheets as a result of imperfections in its production. And in some recent studies, researchers found a freshly cleaved basal surface of pyrolytic graphite exhibits uniform and high electrochemical activity similar to noble metal electrodes for outer-sphere redox processes before the activity decreases significantly over time.
“In any case, from a practical perspective, it seems inefficient to utilize only edge sites due to a low edge to plane ratio in the graphene,” three researchers write in a recent paper. “Thus, other structural imperfections should be used to impart electrocatalytic properties to the whole graphene surface.”
The researchers come from three different institutes in Russia—the Russian Academy of Sciences Joint Institute for High Temperatures (JIHT RAS), the Moscow Institute of Physics and Technology (MIPT), and Skolkovo Institute of Science and Technology. And in the paper, they investigate how different intrinsic and extrinsic defects in graphene influence electron transfer kinetics.
Intrinsic defects are defects formed by a disturbance in the crystal lattice without any additional “alien” atoms. Examples focused on in this study include Stone-Wales defect (rotation of one C-C bond), monovacancy (absence of one carbon atom), and a set of divacancies (absence of two carbon atoms that can relax to different structures).
Extrinsic defects are when the defect contains foreign atoms (in the case of graphene, non-carbon atoms). Examples focused on in this study include substitutional nitrogen atom (change one carbon atom to nitrogen) and epoxy (-O-) and hydroxy (-OH) groups.
To investigate the defects, the researchers used density functional theory to perform atomic and band structure calculations. These calculations were then combined with the Gerischer model for electron transfer kinetic calculations.
In an email, senior researcher of JIHT RAS and MIPT associate professor of physics Sergey Kislenko explains why the Gerischer model works well for performing electron transfer kinetic calculations in graphite-based electrodes.
“To calculate the rate constant of [electron transfer between a graphene electrode and a redox couple], one needs to know a) the distribution of energy states of the electrode, b) the distribution of energy states of the redox couple arising from the interaction with solvent molecules, and c) a method how to describe multiple electron transfers between isoenergetic states in contact phases,” he says. “The Gerischer (or sometimes it’s called Gerischer-Marcus) theory allows performing such calculations.”
Kislenko notes that the Gerischer model contains some simplifications of the system. In particular, the model is applicable for nonadiabatic electron transfer only and omits the structure of a reaction layer in solution and distance dependence of the coupling element H(z), which means the rate constant cannot be calculated for specific points on the surface. However, for estimating the average rate of electron transfer for the surface as a whole, Gerischer provides “a reasonable approximation,” he says.
In the MIPT press release, Kislenko discusses the findings.
“It turned out that introducing defects into a pristine graphene sheet can lead to an increase in the density of electronic states near the Fermi level and catalyze electron transfer,” he explains. “Also, depending on the kind of defect, it affects the density of electronic states across various energy regions in different ways.”
Among the defects, monovacancy demonstrated the most pronounced effect—such defects increased acceleration of electron transfer by an order of magnitude with respect to the pristine graphene.
In the email, Kislenko says they are now using a slightly different approach to overcome the Gerischer simplifications. In particular, they are looking to obtain the electron transfer rate constant near point defects with a spatial resolution, and “the work is already close to publication,” he says.
He says their end goal is to combine the most relevant theoretical approaches into a single multi-factor model that describes the process of electron transfer in detail, thus making directional molecular electrode design feasible.
“Moreover, we want to automate this instrument as much as possible by creating a software in which the whole theory described above will be embedded,” he adds.
The paper, published in Electrochimica Acta, is “Influence of defects in graphene on electron transfer kinetics: The role of the surface electronic structure” (DOI: 10.1016/j.electacta.2020.136011).
Author
Lisa McDonald
CTT Categories
- Basic Science
- Nanomaterials